Contents
A dominant player in the Earth’s climate system
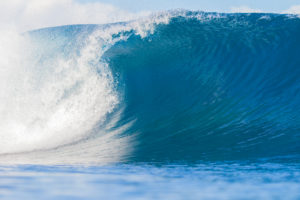
Covering just over 70% of the Earth’s surface, the oceans contain huge volumes of water and, with their high heat capacity and ability to mix both vertically and horizontally, the oceans can store and transport vast amounts of heat. Heat exchange between the ocean and the atmosphere is a major driver in the climate system.
The IPCC estimates that over the last 50 years as much as 93% of the excess heat in the Earth system has been held in the oceans – they have acted as a braking system on climate change by absorbing ~30% of man-made CO2 emissions. The future role of the oceans as the world warms is a topic of great interest to climate scientists.
Their high heat capacity but slower motion in comparison to the atmosphere means that the oceans handle like a giant tanker – huge, powerful and slow to change – but once a change is set in motion, returning to their original state is difficult. Their slower, stabilising effect and longer timescales of change have seen the oceans referred to as the flywheel of the climate system.
Ocean circulation occurs over centuries and a full cycle takes between 1000 to 1500 years – which means the oceans still carry water and climate signals from the Middle Ages (5th – 15th century)! Although the oceans may be relatively slow, they do vary and changes in circulation can induce temporary climate fluctuations today.
Model
The NorESM ocean model is based on the Miami Isopycnic Coordinate Ocean Model (MICOM) and further developed at the Bjerknes Centre in Bergen. The modellers here use it to look more closely at aspects of the ocean such as the exchanges between the North Atlantic and the Arctic, decadal-to-centennial scale climate change and how heat is moved and redistributed.
– Horizontal coordinates –
The horizontal grid of an ocean model is based on degrees of longitude and latitude, but it can get very complicated modelling onto a spherical object.
Take a look at the two globes below – notice the different shapes and sizes of the grid squares across both of the gridding methods, particularly near the poles.
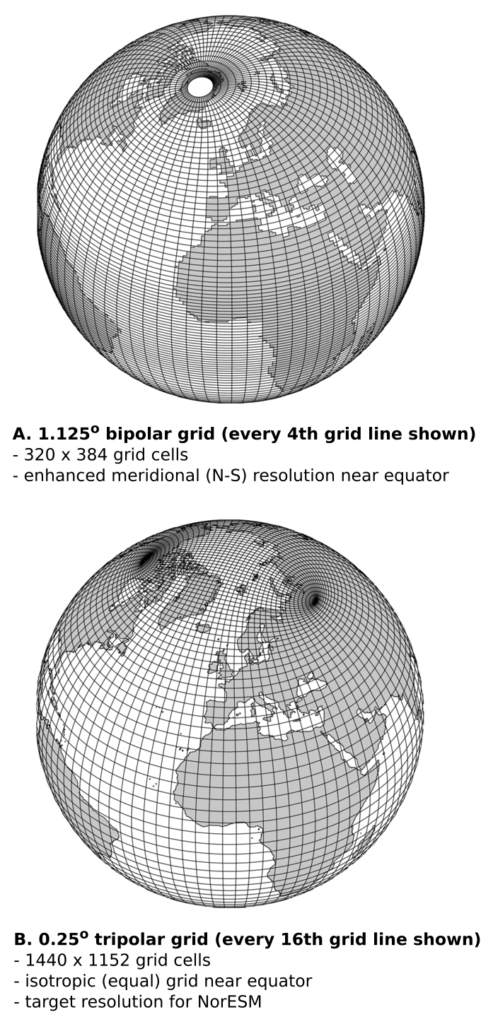
In the bipolar grid at the top, you can see how distorted the grid squares become as they approach the single northern pole. This vanishing point creates a “singularity” – which causes big problems in the maths and quite a headache for the modellers.
The tripolar grid underneath overcomes this by adding in a second pole – see how the distortion is reduced and the grid squares remain more evenly spaced and shaped.
Notice also that the pole – or indeed poles – aren’t actually over the ocean and the real North Pole, but rather the pole(s) have been shifted onto land. Now, since this is the ocean model, the land is of limited interest and distortions or lower resolution here will have no impact. Moving the poles onto land allows ocean modellers to limit the distortions to the grid and maintain resolution over the areas that they are actually interested in – just don’t tell the land modellers!
One of the vital roles of the Coupler is to marry the different ocean, land and atmosphere grids together, so the same grid square in one is talking to its matching grid square in all components in both space and time.
A helpful by-product of the tripolar grid is the improved resolution over the Arctic Ocean – an area of particular interest to modellers and climatologists and considered to be the ‘canary in the coal mine’ in terms of its sensitivity to climate change.
In the Southern Hemisphere, things are a bit easier. The South Pole sits in the middle of a huge continent and this landmass has the effect of absorbing the troublesome mathematical singularity and distortions. Once you get off the continent and out into the Southern Ocean where you can start doing the ocean modelling, you are so far away from the pole the grid has already evened out considerably. So no need for an extra pole here!
The tripolar grid shown here also shows the NorESM ocean model at its highest resolution of 0.25° (the image can only squeeze in showing every 16th grid line!) in comparison to the 1° resolution shown in the bipolar image. Notice how the coastlines have smoothed out and more details such as the small islands in the Atlantic have suddenly appeared. This high resolution tripolar grid is considered state-of-the-art. But it is also very computationally expensive and often the model is run at 1° (as shown here in the bipolar picture) or even 3° resolution, depending on the type of experiment or study the modeller is looking into – and these are considerably less computationally intense.
– Vertical coordinates –
For many models the vertical coordinate is simply depth, but some models, such as the ocean model used in NorESM, use potential density instead.
Potential density has a number of advantages over depth as it is better at representing some of the very complex processes observed in areas such as dense water overflows or beneath ice shelves.
Potential density also does a better job at reproducing the weak but very important vertical mixing that occurs between layers of the ocean, known as isopycnic surfaces (surfaces of equal density) – something that is particularly challenging to model.
Models are evolving alongside our own understanding of the climate system as a whole and the ice component is now getting more and more focus as we come to understand its roles within the system better. Using potential density and the advantages it provides means that ice can be placed upon the same grid, allowing the ocean model to adapt to interact with ice sheets and floating ice shelves.
This means that in the future, NorESM and other similar models will be able to make advances in simulating the mass balance of the ice sheets and improve projections of sea level for studies of both future and past climates.
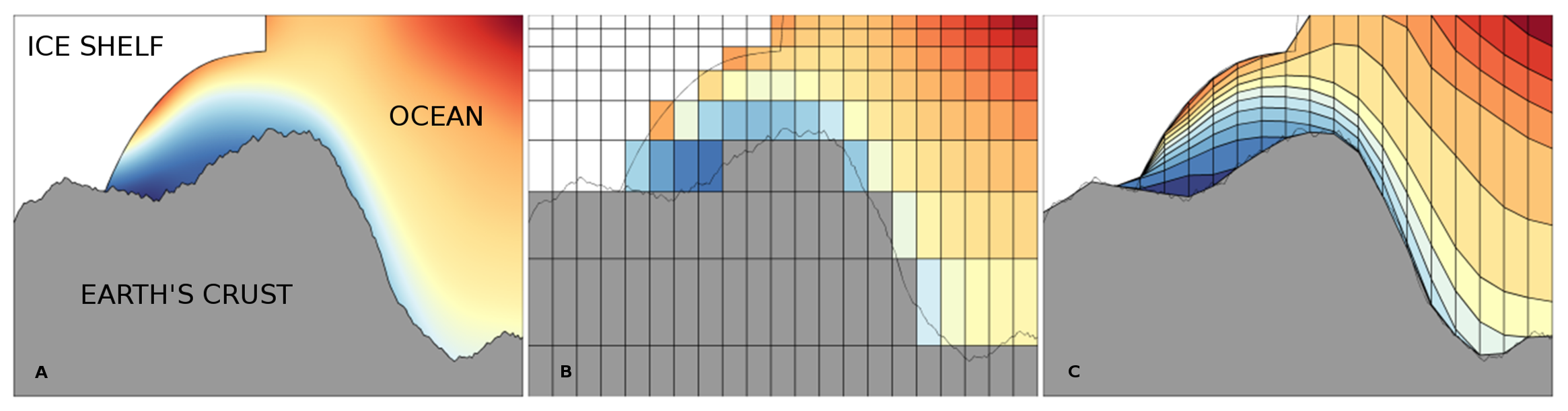
The three pictures above show an example of an area where ice and ocean interact, and how the different choices for the vertical coordinate perform. The left-hand image (A) sets up a typical scenario, showing the vertical cross section of the ice shelf and the ocean, with drainage from the ice shelf providing a continuous production of cold, heavy water shown in blue. The colours grade up to the least dense waters in red at the surface. B and C then compare the model simulations using depth and potential density as the vertical coordinate respectively.
Notice how potential density allows for a more accurate idea of the structure and flow of water masses beneath the ice shelf. For example – look at how the heavy blue water moves: it builds up before overflowing and being rapidly transported into the deep ocean, and flows according to its density, flowing along layers of equal density and then spreading out horizontally, rather than flowing across isopycnals.
Physics
The Ocean model is forced by three fundamental physical principles: at the surface – wind and buoyancy, and in the deeper ocean – tidal currents. The physical processes defined by these make up the basic mathematical equations on which the ocean model is based.
Note the close connection between the surface ocean and atmospheric processes. Often it is information that is provided by the Atmosphere that is used to force the Ocean. This highlights the interconnected and extremely complex nature of Earth System Models and the role of the Coupler in feeding the relevant information between the different components.
– Wind –
The force of atmospheric winds on the surface of the ocean sets up global equations for surface circulation.
As we have seen, atmospheric circulation creates well defined wind patterns. These winds blow across the surface of the ocean, creating a drag that moves the water and causes it to build up in the direction of wind motion.
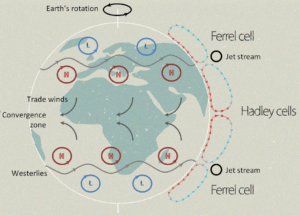
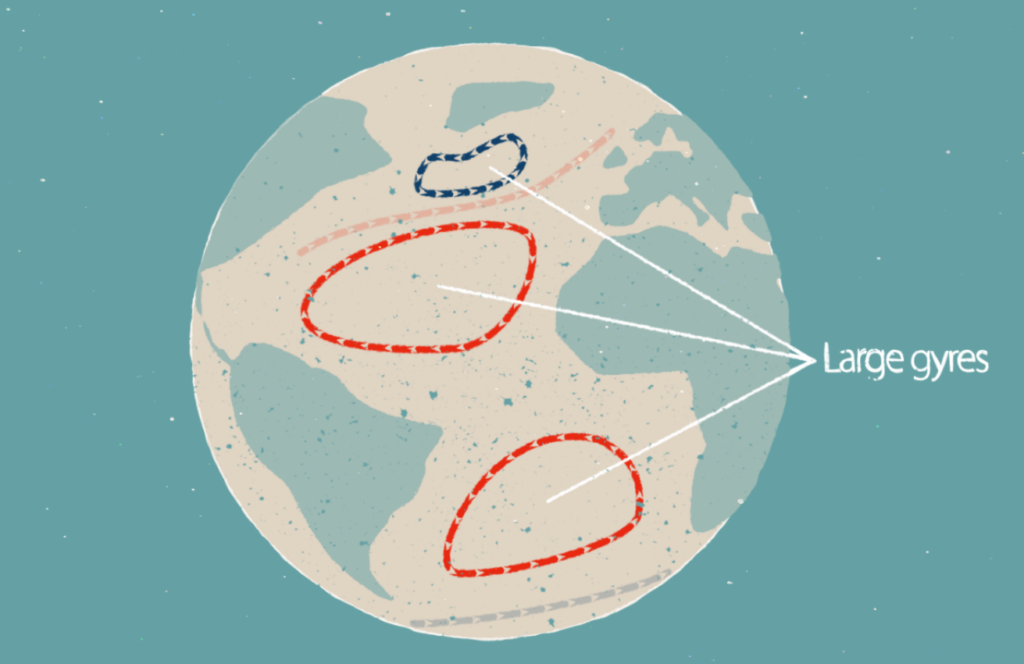
The Coriolis Effect (the Earth’s spin) then begins to have an influence, deflecting ocean currents to the right of the dominant wind direction in the Northern Hemisphere and to the left in the Southern. Continental landmasses and submarine topography also re-route or block currents, helping to create circular flow patterns, known as gyres.
Have a look at the two schematics above. Notice how the dominant ocean circulation reflects the dominant atmospheric wind direction. with the easterly winds in the tropics helping generate the lower west-flowing limb of the gyre in the clockwise moving sub-tropical North Atlantic gyre and the westerly winds of the mid-latitudes reflected in the east-flowing upper limb. The anti-clockwise circulation of the more poleward low pressure atmosperic systems also drives the opposing flow pattern of the sub-polar gyre.
The western sides of the gyres act as the oceans arteries, pumping warm saline water in huge, fast flowing western boundary currents (flowing along the eastern edge of the continents). On the opposite side, weaker eastern boundary currents form the veins, returning cooled water back towards the equator.
– Buoyancy –
At a given depth, water density is determined by two things – its temperature and its salinity.
Warm water is lighter than cold water; fresh (low salinity) water is lighter than salty water. Very cold and salty water is very heavy and will tend to sink.
This balance between temperature and salinity is determined by:
- heat exchange – driven by the difference in heat between the warm equator and the cold poles
- freshwater exchange – the balance between precipitation (which freshens the water mass), and evaporation (which increases salinity).
Explore the effects of different densities by making your own colourful – but completely UNDRINKABLE!! – density cocktail!
You will need:
Baby oil (0.83) Cooking oil (0.92) Fresh water (1.00) Milk (1.03) Washing up liquid (1.06) Blackcurrant cordial (1.33) Honey (1.40)
Numbers give the density of the liquid in kg/L.
Try gently adding an ice cube and see where that sits – or some berries or a piece of cork.
Cocktail masters and photo credit: Petra Langebroeck and Elin Darelius
At their root, these processes are driven by the Sun and the latitudinal difference in incoming radiation and greenhouse gas forcings that occur between the equator and the poles. Much of this happens within the atmosphere and these aspects and their interaction with the ocean are discussed in more detail there. Again, the Coupler plays an important role in connecting these different components.
– Tides –
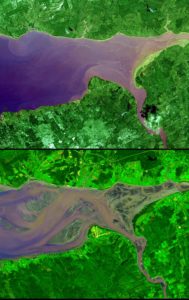
It’s so much easier to appreciate the processes that occur at the surface of the ocean because we actually get to see and feel their effects – it’s easy to forget the deeper ocean, since it’s largely hidden from view. But scientists studying the deep ocean have shown that this unseen region is just as dynamic as the surface. But it’s very hard for modellers to recreate this flow in the models.
The key physical mechanism described in the model equations to create deep ocean flow is the effect of tides on the deep ocean.
Now tides, we know, are generated by the gravitational pull of the moon and the sun on the ocean, and anyone who likes trips to the seaside is familiar with the daily rise and fall of the sea, caused by this gravitational tug.
But there is more to this than just the surface response we can see as we sit with our ice creams on the beach – these tidal effects are felt deep within the ocean and are a key process for generating flow and, as we will see in the Dynamics section below, mixing in the deep. For this reason, tidal phasing is a key element of the model’s basic maths.
Dynamics
– Eddies –
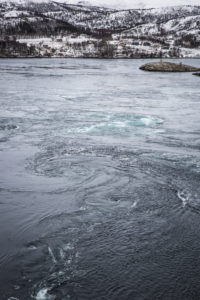
Eddies are the swirling vortices or whirlpools that appear in moving water – you can see mini versions when you drag something through water or as water flows around an obstacle – just, for ocean modellers, these eddies are scaled up quite a bit.
Ocean modellers are interested in the “meso-” or intermediate- scale eddies which are tens to hundreds of kilometers wide and last on the order of a month or more. Mesoscale eddies occur throughout the world ocean and play a vital role in transporting heat in particular, but also other properties such as nutrients, salinity, carbon and oxygen.
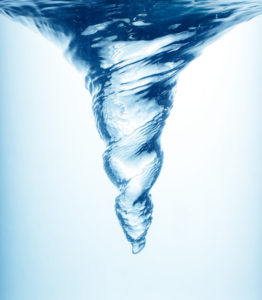
In the ocean, eddies form when water masses flow around obstacles such as islands or seamounts or where strong horizontal gradients (or shear forces) occur along the boundaries of different water masses or currents, creating meanders and instabilities – common features in most of the larger scale ocean currents.
Eddies are energetic features and represent a major component of the ocean’s kinetic energy budget. They flow in a circular motion with a well-defined center which usually contains waters with distinctly different characteristics to the surrounding water mass. The centre can be warm or cold, depending on where the eddy originated from. And they are not just surface features – they can reach deep into the ocean. When they transport waters with high nutrient levels, they are often associated with huge algal blooms.
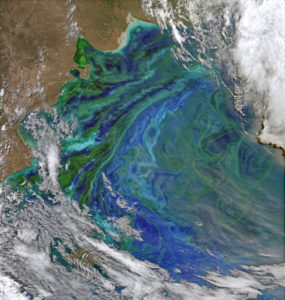
Since eddies play such an important role in distributing heat and other properties throughout the ocean, they must be included in any ocean model. But how a model deals with eddies depends largely on the model’s resolution. The coarser models cannot pick out these eddies, but as resolution improves to 0.25° the models are said to be “eddy permitting” and at 0.1° resolution they are “eddy resolving” – but still only in some areas. Where eddies cannot be resolved their important effects must be parameterised.
Model simulations of the North Atlantic using NorESM at two different resolutions (left: 1° and right: 0.25°). Credit: Mehmet Ilicak
Notice the sharp distinction between the warm subtropical waters and the colder northern waters and how the NAC directs warm waters across to north-west Europe – the waters at the same latitude in North America are far colder. In both model simulations, the NAC is a clearly defined current of water separating the two water masses but it is not until you look at the higher resolution simulation you begin to see some of the details of the current and the endless eddies and turbulence associated with it.
Model simulations of the South Atlantic using NorESM at two different resolutions (left: 1° and right: 0.25°). Credit: Mehmet Ilicak
In the Southern Ocean, the Antarctic Circumpolar Current and South Westerly Winds drive waters in a clockwise direction around Antarctica. However, around the Agulhas region off South Africa, these strong, cold currents meet much warmer currents originating from the Indian Ocean and flowing in the opposite direction. The result is a series of complex fronts – these interact and pinch off parcels of warm Indian waters in huge, spiralling eddies. These are known as Agulhas Rings, and their unique signature can be traced far up into the cooler, fresher surface waters of the Atlantic. This leakage through the Agulhas region plays an important role in regulating the global ocean’s heat and salt distribution. Comparing the two simulations, it is clear that the majority of the flow dynamics are lost at the lower resolution but the higher resolution is able to pick out the large-scale eddies quite well – as well as many subtleties in the flow patterns in the interacting water masses.
– Deep water formation: sinking –
What goes up must come down.
As we have seen, the surface and deep ocean movements create a global ocean circulation. One of the crucial drivers of this circulation is the process of convection, whereby surface waters become dense and sink into the deep ocean. Convection is a key process in both the ocean and the atmosphere.
Although temperature dominates over salinity in the buoyancy equation, to create deep water convection you need both very cold and very saline conditions. There are only a handful of sites in the Earth’s oceans where this process occurs – the Pacific, for example, has no deep water formation because the waters never become saline enough to permit sinking.
The northern North Atlantic, however, is primed for deep water convection. The North Atlantic Current (which you saw in the model simulations above) delivers warm salty water from the tropics into the North Atlantic, which then begins to cool as it continues its journey north. In the winter, heat loss from the surface causes the density of the overlying surface waters to increase and areas begin to sink in isolated plumes. This is aided by localised processes related to the formation of sea ice. As salt water freezes the salt is left behind in a process called brine rejection, causing an increase in the salinity of the surrounding waters. This increased salinity boosts the effect of the falling temperatures and helps drive convection. As the winter progresses these plumes reach further and further down into the deep ocean but as the seasons warm these plumes break down, deep mixing stops and a much thinner mixed layer develops.
Deep water convection is the only mechanism that allows for this direct connection between the atmosphere and the deep ocean. The process allows the deep ocean to breathe, as the sinking surface waters, rich in oxygen from their recent interaction with the atmosphere, permeate the global deep oceans, helping to support life.
– Deep water mixing: rising –
What goes down must come up.
One of the key issues for an ocean model is the need to balance the down-welling of water: the water that gets driven down into the ocean depths must be compensated for by water rising somewhere else.
There are areas of the world’s oceans where this is a relatively simple, wind-driven process: strong winds that push water away or along the coast of a landmass create a circulation pattern that causes cold, deep and often nutrient-rich water to rise up from the deep to replace the water being moved away laterally. This is known as upwelling.
However, upwelling alone is not enough to balance the equation. The missing fundamental piece is deep ocean mixing.
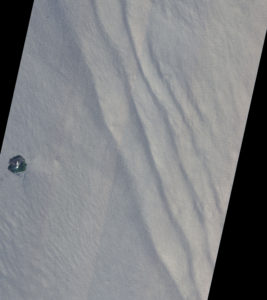
In a layered (or ‘stratified’) ocean, each layer can have very different physical properties. This essentially allows each layer to behave almost independently of those above or below. In the deep ocean, tidal forces pull the water masses over obstacles on the sea floor, causing distortions in the internal layers above and generating waves along these boundaries. Take a look at the simple experiment below – oil overlies water (coloured blue to make the contrast clearer) and the jar is swung like a pendulum. The amount of movement along that internal boundary – especially when the surface stays completely smooth – is striking.
Internal waves are much larger than the ones we are used to seeing on the ocean surface – they can reach hundreds of metres tall and range across tens of kilometres. The huge vertical and horizontal range of these waves, and the turbulent mixing that occurs when they finally break (often many thousands of kilometres away from their source) make them a crucial process in distributing heat, momentum, oxygen, nutrients and other properties throughout the ocean.
Despite their size, and as the experiment below suggests, these waves have little impact on the surface ocean height and are virtually invisible at the water surface. They do, however, have a distinctive tell-tale signature: the upper water layer actually distorts as it flows up and down the huge peaks and troughs of the underlying waves, stretching smooth over the peaks and collecting in the troughs, creating areas of alternating calm and rough surface waters. These different surface characteristics reflect light differently, so from the air or from satellites this contrast is seen as stripes of light (smooth water) and dark (rough water) – the only signs of the monsters lurking beneath. Their vast scale alone hints at their important role within the ocean system, but they are notoriously difficult to study and so to correctly represent in the models.
Playing with internal waves in a glass jar. Also known as the Franklin experiment after Benjamin Franklin noticed this effect in oil lamps whilst onboard a ship to Madeira back in 1761. Credit: Martin King.
Some of the densest waters in the world ocean are generated in the North Atlantic by convection in the Arctic. However, deep ocean mixing is so effective in this region due to its rough seabed topography, that the signature of this extreme density is quickly mixed out of the waters. When scientists map the different water masses in the Atlantic, the deep ocean is actually filled with dense waters originating from Antarctica all the way up into the northern latitudes – because this water is less efficiently mixed, remaining very well defined and very dense.
Biogeochemistry
The ocean is involved in a number of biogeochemical cycles which all involve a close interconnection with the other elements of the other earth system – in particular the atmosphere and the land components. These include oxygen, nitrogen, silicate, DMS (dimethylsulphide) and other nutrient cycling. However, it is the ocean carbon cycle which plays a particularly important role within the climate system.
– Carbon cycle –
The cycling of carbon is one of the most fundamental parts of climate modelling: carbon plays a vital role in life on Earth, from building our own bodies and the plants and animals we need to survive, to sustaining a habitable global climate.
The IPCC states that prior to industrialisation, the oceans contained 60 and 20 times more carbon than the atmosphere and land systems, respectively. The ocean carbon reservoir completely dominates the system. So what happens with the ocean and how it deals with carbon is a big deal to scientists.
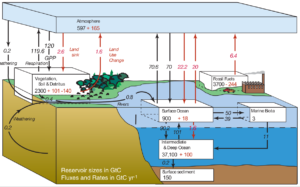
The dominance of the ocean in the global carbon budget is due to chemistry of seawater itself. Carbon in the form of CO2 gas in the atmosphere (weakly acidic) can diffuse easily into seawater (weakly alkaline) and once there it rapidly reacts to form carbonic acid (H2CO3), bicarbonate (HCO3–) and carbonate (CO32-). This series of reactions – known as the marine buffer – controls the pH of the ocean and determines how much CO2 the ocean can absorb – far more than would be expected from solubility alone.
The amount of exchange between the ocean and the atmosphere is largely determined by the concentration gradient and the rate of exchange is largely controlled by wind speed – higher winds are generally associated with greater rates of uptake. Importantly therefore – some regions can be sources and some can be sinks. You can see this wind speed dependence and source (the reds and yellows) vs sink (the blues and purples) pattern in the map below, from the IPCC 4th Assessment Report. Compare these areas of extremes with the patterns of atmospheric circulation.
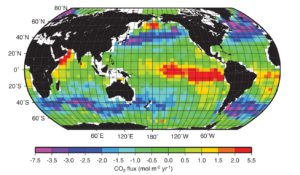
In the ocean, carbon takes three different forms:
DIC: dissolved inorganic carbon – so all the CO2 , H2CO3 , HCO3– and CO32-.
DOC: dissolved organic carbon – so mostly the ultra-fine material produced during decomposition of organic matter and dissolved in the seawater.
POC: particulate organic carbon (living and dead) – so the less dissolved bits of decomposing things as well as all the non-dead things – from whales to plankton.
The carbon can come from within the ocean system itself and also from the land and atmosphere components, such as via river runoff from land or wet deposition from the atmosphere via rainfall.
There are three so-called “pump” systems for carbon in the ocean which drive the exchange of carbon between the ocean and the atmosphere.
The solubility pump
This is the simple physics of how much CO2 seawater can hold in solution. Carbon dioxide is more soluble in cold, fresh water than warm, saline water and at increased pressure. So, the cold fresh areas of the ocean pull CO2 out the atmosphere while the warm saline regions pump it back out. If you remember the role of temperature in determining the buoyancy of a water mass, you’ll remember that these cold water masses – now full of nice fresh atmospheric gases such as CO2 – have a tendency to sink. This drags the CO2 into the deeper ocean, away from the surface and any further interaction with the atmosphere – essentially trapping it. The chemistry of seawater makes it possible for the oceans to store and hold a vast amount of CO2 in this way – but as you can imagine from this simple physical relationship, it is extremely dependent on the state of the ocean and in particular, on its temperature. If the oceans warm, their capacity to take up atmospheric CO2 is reduced. Not a good thing if you’ve been relying on this buffering system to absorb the man-made CO2 we’ve been pumping into the atmosphere …
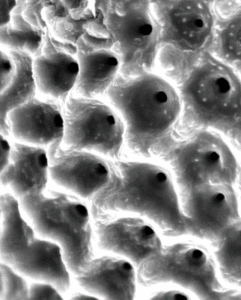
And then there are two biological pumps:
The organic carbon pump
Photosynthesising organisms in the upper ocean use the DIC in the seawater, converting it into organic material. This material then sinks out of the surface waters and into the deeper ocean. A small percentage of this will then go on to actually be buried in the ocean floor and removed from the ocean-atmosphere carbon cycle, except on long-term geological time scales.
The CaCO3 counter-pump
Many marine plankton (such as coccolithophores and foraminifera) make shells from calcium carbonate (CaCO3) which they create from the minerals and elements dissolved in the seawater. The sequence of reactions actually causes a net increase in CO2 in the surface waters. The CaCO3 particles also join the other POC flux to the deeper ocean.
Given a steady state, the solubility pump dominates the oceanic uptake of CO2 but the biological pumps will respond to changing conditions – such as increased nutrients, changes in atmospheric CO2 or ocean acidification.
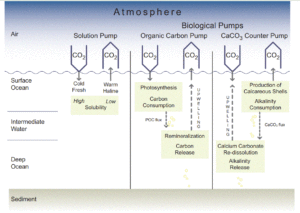
The oceanic carbon cycle and the residence times of carbon within the system works across the full range of timescales: On the scale of years to decades, we have the equilibrium of the surface ocean and atmosphere with respect to CO2 and the cycles of biological productivity. Ocean circulation moving the carbon from the surface into the deep and then back to the surface occurs over the scale of centuries, whilst the amount of carbonate that reaches the sea floor and is preserved or dissolved occurs on a millennial time scale. It is only at such geological time scale (thousands of years) that the burial or release of carbon locked within carbonate rocks becomes relevant for the global carbon cycle.